Abstract
Background
Single-cell RNA sequencing (scRNAseq) data always involves various unwanted variables, which would be able to mask the true signal to identify cell-types. More efficient way of dealing with this issue is to extract low dimension information from high dimensional gene expression data to represent cell-type structure. In the past two years, several powerful matrix factorization tools were developed for scRNAseq data, such as NMF, ZIFA, pCMF and ZINB-WaVE. But the existing approaches either are unable to directly model the raw count of scRNAseq data or are really time-consuming when handling a large number of cells (e.g. n>500).
Results
In this paper, we developed a fast and efficient count-based matrix factorization method (single-cell negative binomial matrix factorization, scNBMF) based on the TensorFlow framework to infer the low dimensional structure of cell types. To make our method scalable, we conducted a series of experiments on three public scRNAseq data sets, brain, embryonic stem, and pancreatic islet. The experimental results show that scNBMF is more powerful to detect cell types and 10 - 100 folds faster than the scRNAseq bespoke tools.
Conclusions
In this paper, we proposed a fast and efficient count-based matrix factorization method, scNBMF, which is more powerful for detecting cell type purposes. A series of experiments were performed on three public scRNAseq data sets. The results show that scNBMF is a more powerful tool in large-scale scRNAseq data analysis. scNBMF was implemented in R and Python, and the source code are freely available at https://github.com/sqsun.
Similar content being viewed by others
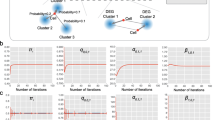
Background
Single-cell RNA-sequencing (scRNAseq) analysis plays an important role in investigating tumour evolution, and is more powerful to characterize the intra-tumor cellular heterogeneity [1, 2]. Compared with traditional RNA sequencing (i.e. bulk RNAseq) which measures the specific gene expression level within a cell population, scRNAseq quantifies the specific gene expression level within only an individual cell [3, 4]. scRNAseq is more likely to understand the detailed biological processes of cell developmental trajectories and cell-to-cell heterogeneity, providing us fresh insights into cell composition, dynamic cell states, and regulatory mechanisms [5–8].
However, there are still several big challenges we have to carefully deal with before analyzing scRNAseq data [9, 10]. The first challenge is that the scRNAseq data is easy to involve some unwanted variables [11, 12], e.g. batch effects, confounding factors, etc. Moreover, the scRNAseq data set has their own characterizes, such as gene expression matrix is extremely sparse because of the quite small number of mRNAs represented in each cell [13]; current sequencing technologies, e.g. CEL-Seq2 [14] and Drop-seq [15], etc, do not have enough power to quantify the actual concentration of mRNAs (i.e. well-known “dropout events”) [16]; the heavy amplifications may result into strong amplification bias [17]; cell cycle state, cell size or other unknown factors may contribute to cell-cell heterogeneity even within the same cell type [18].
The second important feature of the scRNAseq data set is of count nature [19]. In most RNA sequencing studies, the number of reads mapped to a given gene or isoform is often used as an intuitive estimate of its expression level. To account for the count nature of the RNA sequencing data, and the resulting mean-variance dependence, most statistical methods were developed using discrete distributions in differential expression analysis, i.e., PQLseq [20], edgeR/DESeq [21, 22], and MACAU [23]. Therefore, a nature choice of analyzing scRNAseq data is to develop count-based dimensionality reduction methods. Although several dimensionality reduction techniques have been already applied to scRNAseq data analysis, such as principal component analysis (PCA) [24]; independent components analysis (ICA) [25], and diffusion map [26]; partial least squares (PLS) [27, 28]; nonnegative matrix factorization (or factor analysis) [29, 30], gene expression levels are inherently quantified by counts, i.e., count nature of scRNAseq data [31, 32].
Therefore, developing the bespoke scRNAseq dimensionality reduction method has been triggered within the last two years. The first factor analysis method, ZIFA, is trying to model the drop-out events via the zero-inflated model, but the method does not take into account the count nature of the data [33]; pCMF is trying to build sparse Gamma-Poisson factor model within the Bayesian framework, but such method does not include the covariates [34]; ZINB-WaVE is trying to involve both gene-level and sample-level covariates via a hierarchical model, but the method is really time-consuming when sample size is large [35, 36].
Here, in this paper, we propose a fast and efficient count-based matrix factorization method that utilizes the negative binomial distribution to account for the over-dispersion problem of the count nature of scRNAseq data, single-cell Negative Binomial-based Matrix Factorization, scNBMF. The reason of choosing negative binomial model instead of zero-inflated negative binomial model is that not only the most scRNAseq data sets do not show much technical contribution to zero-inflation (Fig. 1a), but also can largely reduce the computation burden in estimating drop-out parameters for each gene. With the stochastic optimization method Adam [37] implemented within TensorFlow framework, scNBMF is roughly 10 – 100 times faster than the existing count-based matrix factorization methods, such as pCMF and ZINB-WaVE. To make the proposed method scalable, we apply scNBMF to analyze three publicly available scRNAseq datasets. The results demonstrate that scNBMF is more efficient and powerful than other matrix factorization methods.
A simple example to show the parameter effect or optimizer effect of NMI and ARI in scRNA-seq data on clustering. a This figure shows the relationship between mean gene expression levels and dropout rates. The black line indicates observed value, which is computed by the number of unexpressed cells divided by the number of cells; The red line represents expected value, which is calculated by negative binomial distribution with mean gene expression levels and dispersion parameter ψ(ψ=mean(ψi))b This figure shows how optimizers affect the performance of different methods on NMI and ARI. c-d These two figure indicate how the number of factors affect the NMI and ARI, respectively
Materials and methods
scNBMF: model and algorithm
scNBMF is to fit the logarithm likelihood function of negative binomial model-based matrix factorization. Given n cells and p genes, we denote Y as a gene expression matrix, and its element yij is the count of gene i and cell j. To account for the over-dispersion problem, we model the gene expression level yij as a random variable following the negative binomial distribution with parameters μij and ϕi, i.e.,
where the rate parameter μij denotes the mean expression level for gene i and cell j; the parameter ϕi represents variance of gene expression, typically means gene-specific over-dispersion; NB is the negative binomial distribution, i.e.
For the rate parameter μij, we consider the following regression model
where Nj is the total read count for the individual cell j (a.k.a read depth or coverage); Wik is the loadings while Hkj is the factors represents the coordinates of the cells, which can be used to identify cell type purpose; K is the pre-defined number of components; When all ϕi→0, the negative binomial distribution will reduce to the standard Poisson distribution.
Therefore, the log-likelihood function for gene i and cell j is
where μ denotes the mean gene expression matrix and its element \(\mu _{ij}=e^{log(N_{j}) + {\sum }_{k = 1}^{K} W_{ik} X_{kj} }\); ϕ is a p-vector, and its element ϕi represents the over-dispersion parameter for gene i.
To make our model more interpretation for the biological applications, we introduce a sparse penalty (LASSO) on loading matrix W since some genes are expressed while some are not in real-world biological processes. Therefore, the objective function of optimization problem becomes
where ∥·∥1 is a l1-norm (i.e. LASSO penalty); λ denotes the penalty parameter.
In the above model, we are interested in extracting the factor matrix H for detecting the cell type purposes. We first estimate the dispersion parameter ϕi) for each gene via edgeR [21] with default parameter settings, then fit the above model using Adam optimizer within TensorFlow. For deep learning model, we set the learning rate of the network as 0.001 and maximum iteration as 18000.
Compared methods and evaluations
To make scNBMF scalable, we compared seven existing methods, i.e. PCA, Nimfa, NMFEM, tSNE, ZIFA, pCMF, and ZINB-WaVE, in the experiments. Since PCA and ZIFA are only for normalized gene expression data, we normalized raw count data following previous recommendations [38]. Typically, we transformed the count data using base 2 and pseudo count 1.0, i.e., log2(Y+1.0), into continuous data. The performance of each method was evaluated by the normalized mutual information (NMI), defined in [39]
and the adjusted rand index (ARI), defined in [40]
where Le and L are the predicted cluster labels and the true labels, respectively; Ke and K are the predicted cluster number and the true cluster number, respectively; nk denotes the number of cells assigned to a specific cluster k (k=1,2,⋯,K); similarly nt denotes the number of cells assigned to cluster t (t=1,2,⋯,Ke); nkt represents the number of cells shared between cluster k and t; and n is the total number of cells.
Public scRNAseq data sets
Three publicly available scRNAseq data sets were collected from three studies:
-
The first scRNAseq data set was collected from human brain [41]. There are 420 cells in eight cell types after excluded hybrid cells including, fetal quiescent cells (110 cells), fetal replicating cells (25 cells), astrocytes cells (62 cells), neuron cells (131 cells), endothelial (20 cells) and oligodendrocyte cells (38 cells) microglia cells(16 cells), and (OPCs, 16 cells), and remain 16,619 genes to test after filtering out the lowly expressed genes. The original data was downloaded from the data repository Gene Expression Omnibus (GEO; GSE67835);
-
The second scRNAseq data set was collected from human pancreatic islet [42]. There are 60 cells in six cell types after excluding undefined cells including alpha cells (18 cells), delta cells (2 cells), pp cells (9 cells), duct cells (8 cells), beta cells (12 cells) and acinar cells (11 cells),and 116,414 genes to test after filtering out the lowly expressed genes. The original data was downloaded from the data repository Gene Expression Omnibus (GEO; GSE73727);
-
The third scRNAseq data set was collected from the human embryonic stem [43]. There are 1018 cells which belong to seven known cell subpopulations that include neuronal progenitor cells (NPCs, 173 cells), definitive endoderm derivative cells (DEDs), endothelial cells (ECs, 105 cells), trophoblast-like cells (TBs, 69 cells), undifferentiated H1(212 cells) and H9(162 cells) ESCs, and fore-skin fibroblasts (HFFs, 159 cells), and contains 17,027 genes to test after filtering step. The original data was downloaded from the data repository Gene Expression Omnibus (GEO; GSE75748).
Results
Model selection
Our first set of experiments is to select the optimization method for the log-likelihood function of negative binomial matrix factorization model. Without loss of generality, we choose the human brain scRNAseq data set. Five optimization methods were compared to optimize the neural networks, i.e., Adam, gradient descent, Adagrad, Momentum and Ftrl. The results show that the Adam significantly outperforms other optimization methods regardless of what criteria we choose (Fig. 1b). Specifically, for NMI, Adam, gradient descent, Adagrad, Momentum, and Ftrl achieve 0.8579, 0.0341, 0.0348, 0.4859, and 0.1251, respectively. Therefore, in the following experiments, we will choose the Adam method to optimize the neural networks.
Our second set of experiments is to select the number of factors in the low dimensional structure of cell types. Without loss of generality, we still choose the human brain scRNAseq data set. We varied the number of factors (k = 4, 6, 10, 15, and 20). The results demonstrate that the number of factors does not impact PCA (Fig. 1c and d; bule line). The other four methods show an increasing pattern when the number of factors varied from 4 to 20 (Fig. 1c and d). Therefore, we choose the top 20 factors in the following experiments.
Public scRNAseq data sets
Our third set of experiments is to apply scNBMF to three scRNAseq real data sets, human brain, human pancreas islet, and human embryonic stem. The cell type information of the three data sets were reported by the original studies. For the comparison, we compared seven other methods, PCA, Nimfa, NMFEM, tSNE, ZIFA, pCMF and ZINB-WaVE. For the evaluation, we extracted the low dimensional structure with top 10 factors, and used k-means clustering method in an unsupervised manner, repeated 100 times to test how well each method can recover the cell type assignments on NMI and ARI in the studies.
The first biological data application is performed on the human brain scRNAseq data set. Figure 2 demonstrates the comparison results of tSNE with respect to seven compared clustering methods. scNBMF shows the clearly cell type patterns with the annotated cell type (Fig. 1h). Also, we carried out the same analysis using PCA (Fig. 2a), Nimfa (Fig. 2b), NMFEM (Fig. 2c), tSNE (Fig. 2d), ZIFA (Fig. 2e), pCMF (Fig. 2f), and ZINB-WaVE (Fig. 2g). For NMI and ARI, scNBMF outperforms the other methods. Specifically, for NMI criterion, PCA, Nimfa, NMFEM, tSNE, ZIFA, pCMF, ZINB-WaVE and scNBMF achieve, 0.582, 0.494, 0.456, 0.712, 0.797, 0.787, 0.892, and 0.901, respectively (Fig. 2i and Table 1); while for ARI criterion, PCA, Nimfa, NMFEM, tSNE, ZIFA, pCMF, ZINB-WaVE and scNBMF achieve, 0.339, 0.258, 0.264, 0.544, 0.721, 0.788, 0.916, and 0.933, respectively (Fig. 2i and Table 1).
Performance evaluation on human brain scRNA-seq data. In this data set there are 420 cells in eight different cell types after the exclusion of hybrid cells. Each kind of color represent a kind of cell type. a-h These eight figures display the clustering output of two dimension of tSNE using eight matrix factorization methods(PCA, Nimfa, NMFEM, tSNE, ZIFA, pCMF, ZINB-WaVE, and scNBMF). f This figure shows NMI and ARI values which are from eight compared methods
The second biological data application is to investigate the character of human pancreas islet scRNAseq data set. This data set has a smaller number of cells - only 60 cells in six cell types. Since all methods do not have enough power to detect the cell type clustering patterns, we did not show the tSNE plots for this data set. For NMI and ARI, tSNE shows the highest performance, while scNBMF achieves the second best performance (Table 1). Specifically, tSNE achieves 0.973 and 0.652 on NMI and ARI, respectively; while scNBMF is 0.716 and 0.472 on NMI and ARI respectively.
The third biological data application is to investigate lineage-specific transcriptomic features at single-cell resolution. To elucidate the distinctions between different lineages, we performed eight matrix factorization methods, i.e., PCA (Fig. 3a), Nimfa (Fig. 3b), NMFEM (Fig. 3c), tSNE (Fig. 3d), ZIFA (Fig. 3e), pCMF (Fig. 3f), ZINB-WaVE (Fig. 3g), and scNBMF (Fig. 3h). scNBMF demonstrates more clearly their respective cell-type patterns compared with other methods. The cell type H1 and H9 show the tight overlapping pattern to indicate the relative homogeneity of human ES cells, such results are also consistence with the previous results [43]. For NMI and ARI, scNBMF outperforms other methods (Fig. 3i and Table 1). Specifically, for NMI, PCA, Nimfa, NMFEM, tSNE, ZIFA, pCMF, ZINB-WaVE and scNBMF achieve, 0.366, 0.414, 0.741, 0.658, 0.888, 0.822, 0.888, and 0.908, respectively; For ARI, PCA, Nimfa, NMFEM, tSNE, ZIFA, pCMF, ZINB-WaVE and scNBMF achieve, 0.187, 0.173, 0.614, 0.538, 0.748, 0.659, 0.721, and 0.763, respectively.
Performance evaluation on human embryonic stem scRNA-seq data set, which contains 1018 cells in seven cell types. Different colors also represent different cell types. a-h These five figure display the clustering output of two dimension of tSNE using five matrix factorization methods(PCA, Nimfa, NMFEM, tSNE, ZIFA, pCMF, ZINB-WaVE, and scNBMF). f This figure shows NMI and ARI values which are from eight compared methods
Computation time
The last set of experiments is to compare the computation time of PCA, Nimfa, NMFEM, tSNE, ZIFA, pCMF, and ZINB-WaVE. Without loss of generality, we use human brain data set to show the computation time of the compared methods (Table 2). Nimfa, NMFEM, ZIFA, pCMF, and ZINB-WaVE are the bespoke scRNAseq methods. Compared with the count-based methods, ZINB-WaVE and pCMF, scNBMF is roughly 100 folds faster than ZINB-WaVE, and 10 folds faster than pCMF. Even comparing the non-count based methods, ZIFA, Nimfa, and NMFEM, scNBMF is still the fastest method.
Conclusion
With rapid developing sequencing technology, a large amount of scRNAseq data sets is easily obtained via different sources. Therefore, computation time is one of these big issues for downstream analysis. On the other hand, scRNAseq data have their own characterizes, i.e., count nature, noisy, and sparsity, etc. These have been triggered the development of a fast and efficient count-based matrix factorization method. In this paper, we proposed a count-based matrix factorization (scNBMF) method to model the raw count data, prevent losing information from normalizing raw count data. On three public biological scRNAseq data sets, scNBMF provides powerful performance compared with other seven methods in terms of NMI, ARI, and computation time.
Zero-inflated distribution is more appropriate method to account for dropouts, e.g. ZIFA and ZINB-WaVE. In current study, we did not consider the zero-inflated model because the tested data sets do not show too much dropouts. However, this is a necessary step in analyzing some scRNAseq data sets. Therefore, we will add the zero-inflated distribution in the future version of the scNBMF.
Biologically, if we incorporate all genes in scRNAseq data analysis, probably it would be able to involve some unwanted variables because not all genes are expressed in biological processes. An interesting direction to improve the performance of scNBMF is to select some informative genes first, this step can largely reduce unwanted variables, and exclude some redundancy genes [44, 45] in the downstream analysis. In addition, because gene expression levels are highly affected by other gene specific annotations, such as GC-content, gene length, and chromatin states [46]. If some interesting variables in the statistical model, such as “drop-out” parameter, can be inferred by annotation information, the method probably will significantly improve the power of detecting cell types from scRNAseq data.
Abbreviations
- ARI:
-
Adjusted rand index
- DESeq:
-
Differential expression
- edgeR:
-
Empirical analysis of digital gene expression data in R
- ICA:
-
Independent components analysis
- MACAU:
-
mixed model association for count data via data augmentation
- NMI:
-
Normalized mutual information
- PCA:
-
Principal component analysis
- pCMF:
-
Probabilistic count matrix factorization
- PLS:
-
Partial least squares
- PQLseq:
-
Penalized quasi-likelihood
- scNBMF:
-
Single-cell negative binomial matrix factorization
- scRNAseq:
-
Single-cell RNA sequencing
- tSNE:
-
t-distributed stochastic neighbor embedding
- ZIFA:
-
Zero-inflated factor analysis
- ZINB-WaVE:
-
Zero-inflated negative binomial-based wanted variation extraction
References
Alexander J, et al. Utility of Single-Cell Genomics in Diagnostic Evaluation of Prostate Cancer. Cancer Res. 2018; 78:348–58.
Love JC. Single-cell sequencing in cancer genomics. Cancer Res. 2015; 75:IA14.
Conesa A, et al.A survey of best practices for RNA-seq data analysis. Genome Biol. 2016; 17:13.
Vieth B, et al.powsimR: power analysis for bulk and single cell RNA-seq experiments. Bioinformatics. 2017; 33:3486–8.
Buettner F, et al.Computational analysis of cell-to-cell heterogeneity in single-cell RNA-sequencing data reveals hidden subpopulations of cells. Nat Biotechnol. 2015; 33:155–60.
Jiang L, et al.GiniClust: detecting rare cell types from single-cell gene expression data with Gini index. Genome Biol. 2016; 17:144.
Kiselev VY, et al.SC3: consensus clustering of single-cell RNA-seq data. Nat Methods. 2017; 14:483–6.
Lonnberg T, et al.Single-cell RNA-seq and computational analysis using temporal mixture modeling resolves T(H)1/T-FH fate bifurcation in malaria. Sci Immunol. 2017; 2:eaal2192.
Wills QF, Mead AJ. Application of single-cell genomics in cancer: promise and challenges. Hum Mol Genet. 2015; 24:R74–R84.
Yuan GC, et al.Challenges and emerging directions in single-cell analysis. Genome Biol. 2017; 18:84.
Ding B, et al.Normalization and noise reduction for single cell RNA-seq experiments. Bioinformatics. 2015; 31:2225–7.
Vallejos CA, et al.Normalizing single-cell RNA sequencing data: challenges and opportunities. Nat Methods. 2017; 14:565–71.
Li WV, Li JYJ. An accurate and robust imputation method scImpute for single-cell RNA-seq data. Nat Commun. 2018; 9:997.
Hashimshony T, et al.CEL-Seq2: sensitive highly-multiplexed single-cell RNA-Seq. Genome Biol. 2016; 17:77.
Macosko EZ, et al.Highly Parallel Genome-wide Expression Profiling of Individual Cells Using Nanoliter Droplets. Cell. 2015; 161:1202–14.
Ziegenhain C, et al.Comparative Analysis of Single-Cell RNA Sequencing Methods. Mol Cell. 2017; 65:631–43.
Brennecke P, et al.Accounting for technical noise in single-cell RNA-seq experiments. Nat Methods. 2013; 10:1093–5.
McDavid A, Finak G, Gottardo R. The contribution of cell cycle to heterogeneity in single-cell RNA-seq data. Nat Biotechnol. 2016; 34:591–3.
Wu AR, Neff NF, Kalisky T, et al.Quantitative assessment of single-cell rna-sequencing methods. Nat Methods. 2014; 11:41–6.
Sun S, Zhu J, Mozaffari S, Ober C, Chen M, Zhou X. Heritability estimation and differential analysis of count data with generalized linear mixed models in genomic sequencing studies. Bioinformatics. 2018. https://doi.org/10.1093/bioinformatics/bty644.
Robinson MD, McCarthy DJ, Smyth GK. edgeR: a Bioconductor package for differential expression analysis of digital gene expression data. Bioinformatics. 2010; 26:139–40.
Anders S, Huber W. Differential expression analysis for sequence count data. Genome Biol. 2010; 11:R106.
Sun S, Hood M, Scott L, Peng Q, Mukherjee S, Tung J, Zhou X. Differential expression analysis for RNAseq using Poisson mixed models. Nucleic Acids Res. 2017; e106:45.
Zurauskiene J, Yau C. pcaReduce: hierarchical clustering of single cell transcriptional profiles. BMC Bioinforma. 2016; 17:140.
Trapnell C, et al.The dynamics and regulators of cell fate decisions are revealed by pseudotemporal ordering of single cells. Nat Biotechnol. 2014; 32:381–U251.
Haghverdi L, Buettner F, Theis FJ. Diffusion maps for high-dimensional single-cell analysis of differentiation data. Bioinformatics. 2015; 31:2989–98.
Chen MJ, Zhou X. Controlling for Confounding Effects in Single Cell RNA Sequencing Studies Using both Control and Target Genes. Sci Rep. 2017; 7:13587.
Sun SQ, Peng QK, Shakoor A. A Kernel-Based Multivariate Feature Selection Method for Microarray Data Classification. Plos ONE. 2014; 9:e102541.
Shao CX, Hofer T. Robust classification of single-cell transcriptome data by nonnegative matrix factorization. Bioinformatics. 2017; 33:235–42.
Zhu X, et al.Detecting heterogeneity in single-cell RNA-Seq data by non-negative matrix factorization. Peerj. 2017; e2888:5.
Miao Z, et al.DEsingle for detecting three types of differential expression in single-cell RNA-seq data. Bioinformatics. 2018; 34:3223–4.
Streets AM, Huang YY. How deep is enough in single-cell RNA-seq. Nat Biotechnol. 2014; 32:1005–6.
Pierson E, Yau C. ZIFA: Dimensionality reduction for zero-inflated single-cell gene expression analysis. Genome Biol. 2015; 16:241.
Durif G, et al.Probabilistic Count Matrix Factorization for Single Cell Expression Data Analysis. BioRxiv; 2017.
Risso D, et al.A general and flexible method for signal extraction from single-cell RNA-seq data. Nat Commun. 2018; 9:284.
Van den Berge K, et al.Observation weights unlock bulk RNA-seq tools for zero inflation and single-cell applications. Genome Biol. 2018; 19:24.
Kingma DP, Ba J. Adam: A Method for Stochastic Optimization. the 3rd International Conference for Learning Representations. San Diego; 2015.
Lin PJ, Troup M, Ho JWK. CIDR: Ultrafast and accurate clustering through imputation for single-cell RNA-seq data. Genome Biol. 2017; 18:59.
Ghosh J, Acharya A. Cluster ensembles. Adv Rev. 2011; 4:305–15.
Hubert L, Arabie P. Comparing partitions. J Classif. 1985; 2:193–218.
Darmanis S, et al.A survey of human brain transcriptome diversity at the single cell level. P Natl Acad Sci USA. 2015; 112:7285–90.
Li J, et al.Single-cell transcriptomes reveal characteristic features of human pancreatic islet cell types. Embo Rep. 2016; 17:178–87.
Chu LF, et al.Single-cell RNA-seq reveals novel regulators of human embryonic stem cell differentiation to definitive endoderm. Genome Biol. 2016; 17:173.
Feng Z, Wang Y. Elf: extract landmark features by optimizing topology maintenance, redundancy, and specificity. IEEE ACM T Comput BI. 2018; 99:1.
Sun S, Peng Q, Zhang X. Global feature selection from microarray data using Lagrange multipliers. Knowl-Based Syst. 2016; 110:267–74.
Sun S, Sun X, Zheng Y. Higher-order partial least squares for predicting gene expression levels from chromatin states. BMC Bioinforma. 2018; 19:113.
Acknowledgements
No applicable.
Funding
Publication of this artical was sponsored by the Top International University Visiting Program for Outstanding Young scholars of Northwestern Polytechnical University; Fundamental Research Funds for the Central Universities (Grant: 3102017OQD098); Natural Science Foundation of China (NFSC; Grants: 61772426 and 61332014).
Availability of data and materials
scNBMF was implemented by R and Python, and the source code are freely available at https://github.com/sqsun. The three publicly scRNAseq datasets are available at https://www.ncbi.nlm.nih.gov/geo/query/acc.cgi?acc=GSE67835https://www.ncbi.nlm.nih.gov/geo/query/acc.cgi?acc=GSE73727https://www.ncbi.nlm.nih.gov/geo/query/acc.cgi?acc=GSE75748
About this supplement
This article has been published as part of BMC Systems Biology Volume 13 Supplement 2, 2019: Selected articles from the 17th Asia Pacific Bioinformatics Conference (APBC 2019): systems biology. The full contents of the supplement are available online at https://bmcsystbiol.biomedcentral.com/articles/supplements/volume-13-supplement-2.
Author information
Authors and Affiliations
Contributions
SS, YC, YL and XS conceived and wrote the manuscript. SS and YC implemented the software and analyzed the data. All authors read and approved the final manuscript.
Corresponding author
Ethics declarations
Ethics approval and consent to participate
No applicable.
Consent for publication
No applicable.
Competing interests
The authors declare that they have no competing interests.
Publisher’s Note
Springer Nature remains neutral with regard to jurisdictional claims in published maps and institutional affiliations.
Rights and permissions
Open Access This article is distributed under the terms of the Creative Commons Attribution 4.0 International License (http://creativecommons.org/licenses/by/4.0/), which permits unrestricted use, distribution, and reproduction in any medium, provided you give appropriate credit to the original author(s) and the source, provide a link to the Creative Commons license, and indicate if changes were made. The Creative Commons Public Domain Dedication waiver(http://creativecommons.org/publicdomain/zero/1.0/) applies to the data made available in this article, unless otherwise stated.
About this article
Cite this article
Sun, S., Chen, Y., Liu, Y. et al. A fast and efficient count-based matrix factorization method for detecting cell types from single-cell RNAseq data. BMC Syst Biol 13 (Suppl 2), 28 (2019). https://doi.org/10.1186/s12918-019-0699-6
Published:
DOI: https://doi.org/10.1186/s12918-019-0699-6